PPPL’s new plasma simulation method promises to transform computer chip manufacturing and fusion energy research.
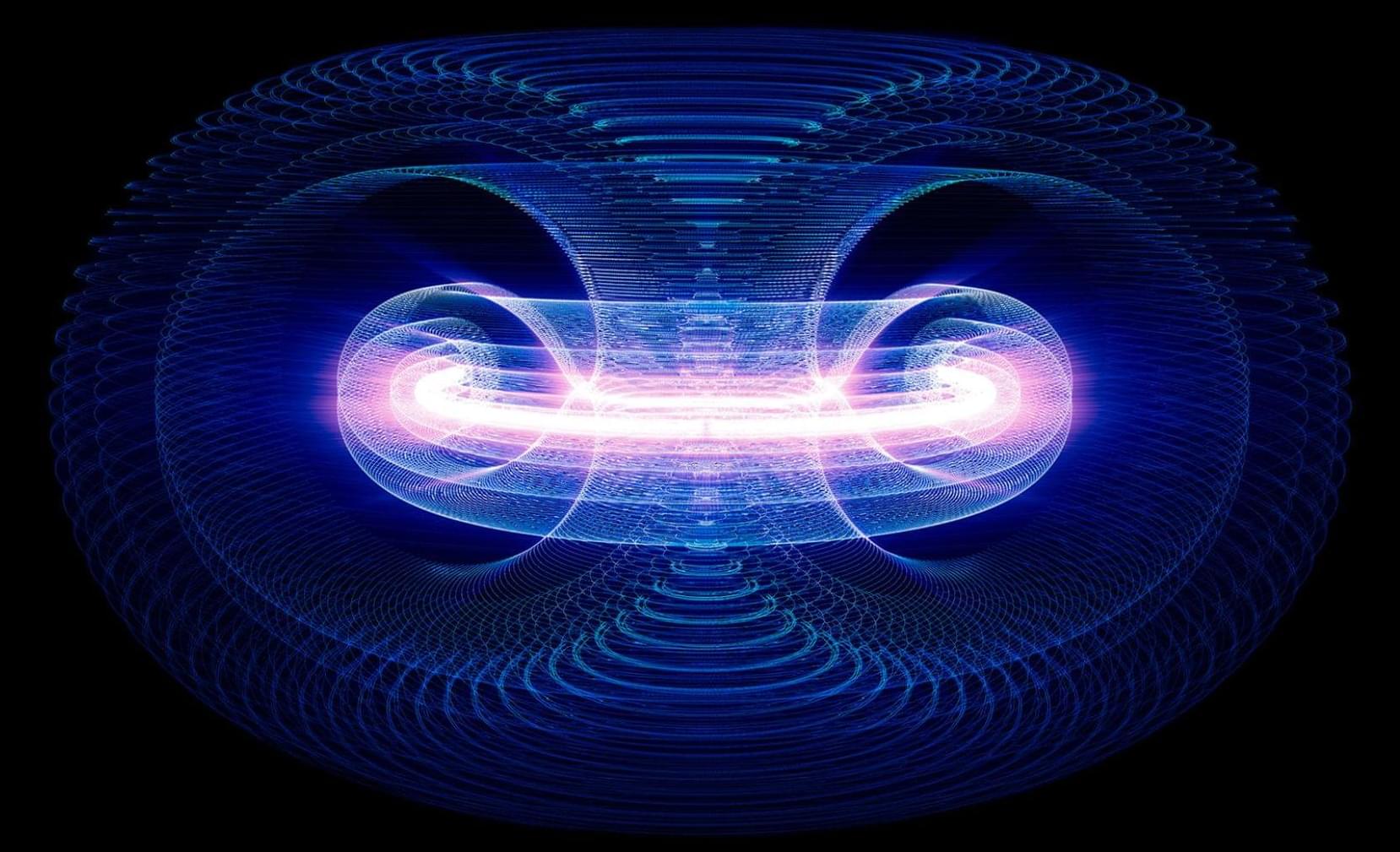
An analysis using unprecedented satellite observations reveals important information about how electrons get heated throughout the Universe.
What connects solar flares that induce space weather, geomagnetic storms that cause auroras, and magnetic disruptions that spoil confinement in magnetically confined fusion devices? All these events rapidly convert stored magnetic energy into kinetic energy of surrounding electrons and positively charged ions in the plasma state of matter. The energy conversion occurs via a fundamental process called magnetic reconnection [1]. But some aspects of reconnection remain poorly understood, despite decades of scrutiny through theoretical studies, ground-and satellite-based observations, lab experiments, and numerical simulations [2]. A key unresolved problem is determining how much of the released magnetic energy goes to the electrons and how much goes to the ions, and by what physical mechanisms this energization occurs.
A US startup is looking to our closest satellite to fill a resources gap here on Earth. Helium-3 is rare on terra firma, but is thought to be abundant in the regolith of the Moon. Interlune has now revealed a full-scale excavator prototype that forms a key component of its lunar Harvester.
The shortage of helium-3 – a stable isotope of helium important for applications ranging from energy production to medical research – was first identified in the US toward the middle of 2008. The US government officially recognized the issue in early 2009, and mitigation efforts put in place.
“The United States supply of 3He comes from the decay of tritium (3H), which the Nation had in large quantities because of our nuclear weapons complex; however, the tritium stockpile has declined in recent years through radioactive decay and is expected to decline in the future because of reduced demand for tritium,” read the intro to a National Isotope Development Center newsletter from 2014.
A research team from the University of South China has developed a set of algorithms to help optimize radiation-shielding design for new types of nuclear reactors.
Their achievement, which was published in the journal of Nuclear Science and Techniques and shared by TechXplore, will help engineers meet the difficult demands for next-gen reactors, including transportable models, as well as those intended for marine and space environments.
Safety is of paramount concern when it comes to nuclear energy, especially considering the public’s perception of this clean energy source following some notable accidents over the past 68 years.
Monte Carlo methods, or Monte Carlo experiments, are a broad class of computational algorithms that rely on repeated random sampling to obtain numerical results. The underlying concept is to use randomness to solve problems that might be deterministic in principle. The name comes from the Monte Carlo Casino in Monaco, where the primary developer of the method, mathematician Stanisław Ulam, was inspired by his uncle’s gambling habits.
Monte Carlo methods are mainly used in three distinct problem classes: optimization, numerical integration, and generating draws from a probability distribution. They can also be used to model phenomena with significant uncertainty in inputs, such as calculating the risk of a nuclear power plant failure. Monte Carlo methods are often implemented using computer simulations, and they can provide approximate solutions to problems that are otherwise intractable or too complex to analyze mathematically.
Monte Carlo methods are widely used in various fields of science, engineering, and mathematics, such as physics, chemistry, biology, statistics, artificial intelligence, finance, and cryptography. They have also been applied to social sciences, such as sociology, psychology, and political science. Monte Carlo methods have been recognized as one of the most important and influential ideas of the 20th century, and they have enabled many scientific and technological breakthroughs.
IN A NUTSHELL 🔬 PPPL’s new simulation method revolutionizes fusion research and chip manufacturing by accurately modeling plasma behaviors. 💻 The development addresses significant computational challenges, enhancing stability and efficiency in plasma simulations. ⚡ Improved simulations allow for precise conservation of energy, ensuring results reflect real-world physical processes. 🚀 Future applications include advancements in fusion
Experience Sunbird and step into the future of propulsion. Dive into our interactive 3D experience and see Sunbird in action as it unlocks new frontiers.
Meet Sunbird, a marvel of space propulsion innovation, powered by our state-of-the-art Dual Direct Fusion Drive (DDFD). With a remarkable specific impulse of 10,000–15,000 seconds and 2 MW of power, Sunbird is redefining what’s possible in space travel.
The compact DDFD isn’t just an engine, it’s a leap forward, providing both thrust and electrical power for next-generation spacecraft. This breakthrough opens unprecedented possibilities to explore the solar system faster and with greater payloads than ever before.
Ready to see the future launch?
A special thank you to Dark Star Labs for bringing our vision to life with this incredible video.
Scientists discover fungus species in Chernobyl nuclear zone have mutated to feed on radiation:
Cryptococcus neoformans, discovered at the site in 1991, feeds on radiation through a process called radiosynthesis. Its high levels of melanin absorb harmful radiation and convert it into chemical energy, much like how plants use photosynthesis to create energy.
NASA scientists, in collaboration with Johns Hopkins University, are now testing melanin extracted from the fungi aboard the International Space Station. ’ If successful, this natural shield could protect astronauts and equipment from cosmic rays, a significant challenge for long-term space exploration. “Space radiation is dangerous and damages matter,” explains researcher Radamés J.B. Cordero. “A material like this could shield astronauts and benefit people here on Earth.” This discovery turns a remnant of a nuclear disaster into a potential lifesaver for humanity’s journey into the cosmos.
Learn more.
Radiotrophic fungi are fungi that can perform the hypothetical biological process called radiosynthesis, which means using ionizing radiation as an energy source to drive metabolism. It has been claimed that radiotrophic fungi have been found in extreme environments such as in the Chernobyl Nuclear Power Plant.
Most radiotrophic fungi use melanin in some capacity to survive. [ 1 ] The process of using radiation and melanin for energy has been termed radiosynthesis, and is thought to be analogous to anaerobic respiration. [ 2 ] However, it is not known if multi-step processes such as photosynthesis or chemosynthesis are used in radiosynthesis or even if radiosynthesis exists in living organisms.
Join our free newsletter for weekly updates on the latest innovations improving our lives and shaping our future, and don’t miss this cool list of easy ways to help yourself while helping the planet.
First appeared on The Cool Down.