Little powerhouses might someday replace billion-dollar, kilometer-long behemoths at major x-ray facilities.
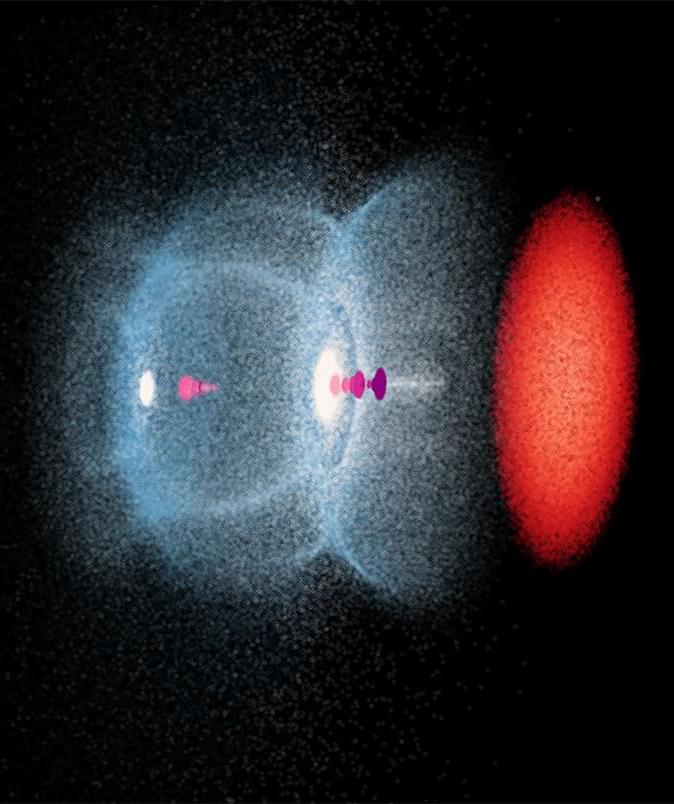
In a ground-breaking theoretical study, two physicists have identified a new class of quasiparticle called the paraparticle. Their calculations suggest that paraparticles exhibit quantum properties that are fundamentally different from those of familiar bosons and fermions, such as photons and electrons respectively.
Using advanced mathematical techniques, Kaden Hazzard at Rice University in the US and his former graduate student Zhiyuan Wang, now at the Max Planck Institute of Quantum Optics in Germany, have meticulously analysed the mathematical properties of paraparticles and proposed a real physical system that could exhibit paraparticle behaviour.
“Our main finding is that it is possible for particles to have exchange statistics different from those of fermions or bosons, while still satisfying the important physical principles of locality and causality,” Hazzard explains.
Interactions between atoms and light rule the behavior of our physical world, but at the same time, can be extremely complex. Understanding and harnessing them is one of the major challenges for the development of quantum technologies.
To understand light-mediated interactions between atoms, it is common to isolate only two atomic levels, a ground level and an excited level, and view the atoms as tiny antennas with two poles that talk to each other. So, when an atom in a crystal lattice array is prepared in the excited state, it relaxes back to the ground state after some time by emitting a photon.
The emitted photon does not necessarily escape from the array, but instead, it can become absorbed by another ground-state atom, which then gets excited. Such an exchange of excitations, also referred to as dipole-dipole interaction, is key for making atoms interact, even when they cannot bump into each other.
A research team has discovered that achiral hard banana-shaped particles can spontaneously form exotic structures like skyrmions and blue phase III phases. Skyrmions are tiny vortex-like structures found in various condensed-matter systems, such as helical ferromagnets and liquid crystals. Blue phase III is an amorphous phase of liquid crystals that possesses strong optical activity. Achiral particles are particles that can be superimposed on their mirror image. The team’s findings have potential applications in photonics and memory devices.
Their work was published in Nature Communications on August 8, 2024.
Skyrmions typically arise from chiral interactions, that is, a molecular interaction that occurs between molecules that both possess chirality. Molecules with chirality cannot be superimposed on their mirror images. British physicist Tony Skyrme introduced skyrmions in 1961.
A collaborative team of researchers from GSI/FAIR, Johannes Gutenberg University Mainz, and the Helmholtz Institute Mainz has advanced our understanding of the “island of stability” in superheavy nuclides. They achieved this by precisely measuring the superheavy rutherfordium-252 nucleus, now identified as the shortest-lived superheavy nucleus on record. Their findings were published in Physical Review Letters
<em> Physical Review Letters (PRL)</em> is a prestigious peer-reviewed scientific journal published by the American Physical Society. Launched in 1958, it is renowned for its swift publication of short reports on significant fundamental research in all fields of physics. PRL serves as a venue for researchers to quickly share groundbreaking and innovative findings that can potentially shift or enhance understanding in areas such as particle physics, quantum mechanics, relativity, and condensed matter physics. The journal is highly regarded in the scientific community for its rigorous peer review process and its focus on high-impact papers that often provide foundational insights within the field of physics.
A research team has discovered that by using a new method of “atomic spray painting,” they can tweak the atomic structure of lead-free potassium niobate in order to enhance its ferroelectric properties.
The study, created by a team led by Penn State researchers, explains how molecular beam epitaxy can be employed to deposit atomic layers onto a substrate to create thin films, as a report by SciTechDaily explained.
Using a technique called strain tuning, the researchers adjusted how successive layers are aligned to modify a material’s properties by stretching or compressing the atoms that make up its crystal structure.
Experiments conducted at Montana State University in collaboration with Columbia University and the Honda Research Institute have resulted in the emission of single photons of light in a new type of quantum material—a feat that could lead to the development of controllable light sources for use in quantum technologies.
A comprehensive article about the breakthrough was published in the journal Nature Communications. It describes ultra small, two-dimensional, ribbon-shaped materials measuring one atom thick and tens of atoms wide—about a thousand times narrower than the width of a human hair.
The nanoribbons were grown by the Honda Research Institute, stretched over specialized surfaces developed by Columbia to stimulate photon emission, then manipulated and tested by the MSU team, which analyzed and described the nanoribbons’ characteristics, including their ability to emit single photons.
In a landmark achievement, an international team of researchers has successfully engineered the world’s first ideal Weyl semimetal, a quantum crystal that exhibits exotic electromagnetic properties. This innovative material, synthesized from a topological semiconductor, hosts a single pair of Weyl fermions without any irrelevant electronic states, paving the way for potential applications in terahertz devices, high-performance sensors, and low-power electronics.
The discovery, published in Nature, marks a major milestone in the decade-long pursuit of quantum materials, where researchers have been hindered by the presence of undesired electrons that obscure the unique properties of Weyl fermions. By revisiting a theoretically proposed strategy from 2011, the team has created a semimetal with a vanishing energy gap, enabling it to absorb low-frequency light and unlocking new possibilities for optoelectronics and quantum technology.
Go to https://ground.news/startalk to stay fully informed on the latest Space and Science news. Subscribe through our link for 50% off unlimited access to the Vantage plan this month.
Could you travel back in time through a wormhole? Neil deGrasse Tyson sits down with theoretical physicist and Nobel Laureate Kip Thorne to reflect on discovering gravitational waves with LIGO, the science in the movie Interstellar, black holes, and many more mysteries still yet to be answered.
Discover the origin story of the movie Interstellar on its 10th anniversary. Kip explains how science, not fiction, shaped the film’s narrative—from the colossal waves on Miller’s planet to the physics behind black hole time dilation. Discover the recipe for how to create a wormhole and how turning on a time machine could cause it to self-destruct. Plus, learn about the Casimir effect, exotic particles, and how LIGO manipulated vacuum fluctuations to bypass the uncertainty principle.
Neil and Kip dig into the origins of gravitational wave detection, tracing its roots to Joe Weber’s early experiments and Ray Weiss’s unpublished paper. Kip reflects on the decades of work required to make LIGO a success, the challenges of measuring distortions a fraction of a proton’s width, and the historic detection of gravitational waves in 2016 that confirmed Einstein’s predictions.
Why don’t quantum physics and the theory of relativity mix? We discuss the mysteries of quantum gravity, the paradox of black hole information loss, and Kip’s legendary bet with Stephen Hawking and John Preskell. Kip explains why backward time travel may be possible, Hawking Radiation, and theories for why information can be lost. As they explore the intersection of science and art, Kip discusses his passion for storytelling and some of his future projects, from his poetry-art collaborations to documenting the history of LIGO.
Timestamps:
How do particles get mass? Neil deGrasse Tyson and comedian Chuck Nice discover squarks, sneutrinos, the Higgs boson, and whether dark matter has a particle with theoretical physicist Brian Greene.
Go to https://ground.news/startalk to stay fully informed on Space and Science news. Save 40% off through my link for unlimited access to the Vantage plan this month.
Can we finally get to the bottom of what happens when a quark falls into a black hole? Learn about the ultraviolet catastrophe, the start of quantum physics, and Max Planck quantizing packets of energy. We also discuss how Einstein won the Nobel prize for the discovery for which he is least famous.
We take a deep dive into the Higgs boson. Who’s Higgs? What’s a boson? Find out about how the Higgs field creates mass, the different quantum particles, and how quarks create protons and neutrons. Brian breaks down the theory of supersymmetry: does every particle have a counterpart? Learn about squarks, sneutrinos, and whether supersymmetry can give an answer to what dark matter is.
Is the fabric of spacetime woven by tiny wormholes? Discover the Casimir force, quantum fluctuations, and why you need so many dimensions in a string theory universe. We discuss whether the cosmological constant is, in fact, constant. Plus, find out about the biggest mismatch between theory and experiment in physics.
Timestamps: