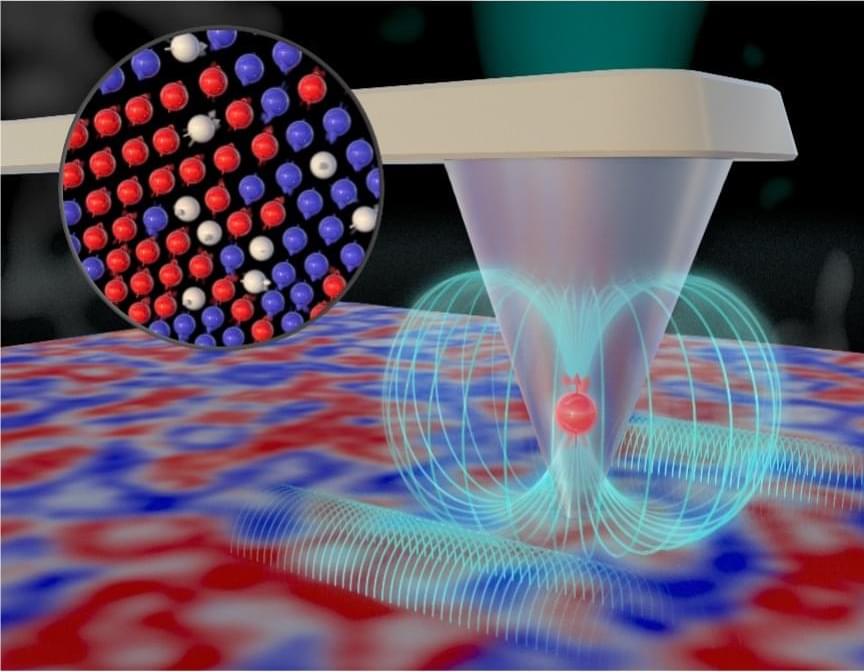
Working at nanoscale dimensions, billionths of a meter in size, a team of scientists led by the Department of Energy’s Oak Ridge National Laboratory revealed a new way to measure high-speed fluctuations in magnetic materials. Knowledge obtained by these new measurements, published in Nano Letters, could be used to advance technologies ranging from traditional computing to the emerging field of quantum computing.
Many materials undergo phase transitions characterized by temperature-dependent stepwise changes of important fundamental properties. Understanding materials’ behavior near a critical transition temperature is key to developing new technologies that take advantage of unique physical properties. In this study, the team used a nanoscale quantum sensor to measure spin fluctuations near a phase transition in a magnetic thin film. Thin films with magnetic properties at room temperature are essential for data storage, sensors and electronic devices because their magnetic properties can be precisely controlled and manipulated.
The team used a specialized instrument called a scanning nitrogen-vacancy center microscope at the Center for Nanophase Materials Sciences, a DOE Office of Science user facility at ORNL. A nitrogen-vacancy center is an atomic-scale defect in diamond where a nitrogen atom takes the place of a carbon atom, and a neighboring carbon atom is missing, creating a special configuration of quantum spin states. In a nitrogen-vacancy center microscope, the defect reacts to static and fluctuating magnetic fields, allowing scientists to detect signals on a single spin level to examine nanoscale structures.