Gravity measurement benefits from optical lattice.
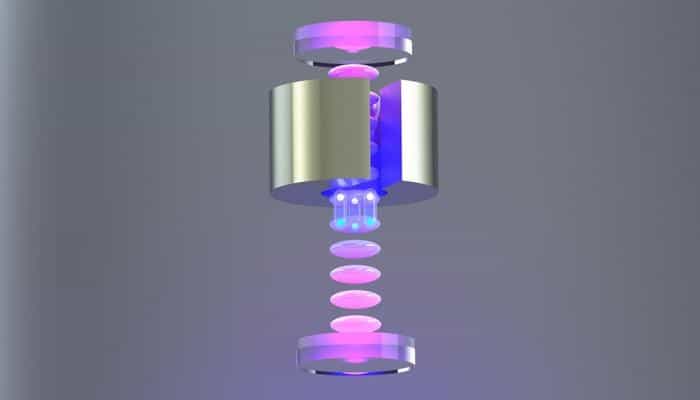
When atomic nuclei and subatomic particles interact, the results are incredibly complex. These are the “many body problems” of quantum mechanics. To help make sense of these interactions, scientists create ways to simplify the range of possible outcomes.
One example is “effective interactions,” which simplify the interactions between a nucleon (a proton or a neutron) and an atomic nucleus. Effective interactions help scientists develop theories of the reactions that result when nuclei collide with each other or with subatomic particles.
These tools are part of a group of methods called effective field theory (EFT). EFT in turn is a type of approach called “ab initio,” or “first principles.” Ab initio means a calculation starts with the established laws of physics without any other assumptions.
Around 80% of the universe’s matter is dark, meaning it is invisible. Despite being imperceptible, dark matter constantly streams through us at a rate of trillions of particles per second. We know it exists due to its gravitational effects, yet direct detection has remained elusive.
Researchers from Lancaster University, the University of Oxford, and Royal Holloway, University of London, are leveraging cutting-edge quantum technologies to build the most sensitive dark matter detectors to date. Their project, titled “A Quantum View of the Invisible Universe,” is featured at the Royal Society’s Summer Science Exhibition. Related research is also published in the Journal of Low Temperature Physics
The team includes Dr. Michael Thompson, Professor Edward Laird, Dr. Dmitry Zmeev, and Dr. Samuli Autti from Lancaster, Professor Jocelyn Monroe from Oxford, and Professor Andrew Casey from RHUL.
How can so many numbers of nature—the constants and relationships of physics—be so spot-on perfect for humans to exist? Coincidence and luck seem wildly unlikely. This question causes controversy, among scientists and among philosophers. Beware: there is more than one answer lurking here.
Free access to Closer to Truth’s library of 5,000 videos: http://bit.ly/376lkKN
Watch more interviews on fine tuning: https://bit.ly/3EyyJQh.
Steven Weinberg is an American theoretical physicist and Nobel laureate in Physics for his contributions to the unification of the weak force and electromagnetic interaction between elementary particles.
Register for free at CTT.com for subscriber-only exclusives: http://bit.ly/2GXmFsP
Closer to Truth, hosted by Robert Lawrence Kuhn and directed by Peter Getzels, presents the world’s greatest thinkers exploring humanity’s deepest questions. Discover fundamental issues of existence. Engage new and diverse ways of thinking. Appreciate intense debates. Share your own opinions. Seek your own answers.
A crystal is an arrangement of atoms that repeats itself in space, in regular intervals: At every point, the crystal looks exactly the same. In 2012, Nobel Prize winner Frank Wilczek raised the question: Could there also be a time crystal—an object that repeats itself not in space but in time? And could it be possible that a periodic rhythm emerges, even though no specific rhythm is imposed on the system and the interaction between the particles is completely independent of time?
For years, Frank Wilczek’s idea has caused much controversy. Some considered time crystals to be impossible in principle, while others tried to find loopholes and realize time crystals under certain special conditions.
Now, a particularly spectacular kind of time crystal has successfully been created at Tsinghua University in China, with the support from TU Wien in Austria.
Kamiokande-II saw the first supernova neutrinos from the famous SN 1987A.
Every few seconds, somewhere in the observable Universe, a massive star collapses and unleashes a supernova explosion. Japan’s Super-Kamiokande observatory might now be collecting a steady trickle of neutrinos from those cataclysms, physicists say — amounting to a few detections a year.
These tiny subatomic particles are central to understanding what goes on inside a supernova: because they zip out of the star’s collapsing core and across space, they can provide information about any potentially new physics that occur under extreme conditions.
At last month’s Neutrino 2024 conference in Milan, Italy, Masayuki Harada, a physicist at the University of Tokyo, revealed that the first hints of supernova neutrinos seem to be emerging from the cacophony of particles that the Super-Kamiokande detector collects every day from other sources, such as cosmic rays hitting the atmosphere and nuclear fusion in the Sun’s core. The result “indicates that we started observing a signal”, says Masayuki Nakahata, a physicist at the University of Tokyo and spokesperson for the experiment, which is commonly referred to as Super-K. But Nakahata cautions that the supporting data — collected over 956 days of observation — are still very weak.
Scientists have developed a new way to trap small particles with light. Building on the Nobel Prize winning technique of optical tweezers (Arthur Ashkin, 2018), a team of physicists, led by Dr. David Phillips at the University of Exeter, has advanced the possibilities of optical trapping.
The research paper, published in the journal Science Advances, is titled “Photon-efficient optical tweezers via wavefront shaping.”
Conventional optical tweezers, developed in the 1980s, are a tightly focused laser beam which can attract and trap certain micro-sized particles or organisms, akin to grabbing something with a pair of tweezers.
A levitating microparticle is observed to recoil when a nucleus embedded in the particle decays—opening the door to future searches of invisible decay products.
For centuries, physicists have exploited momentum conservation as a powerful means to analyze dynamical processes, from billiard-ball collisions to galaxy formation to subatomic particle creation in accelerators. David Moore and his research team at Yale University have now put this approach to work in a new setting: they used momentum conservation to determine when a radioactive atom emitted a single helium nucleus, known as an alpha particle (Fig. 1) [1]. The demonstration suggests that—with further improvements—researchers might be able to use this technique to detect other nuclear-decay products, such as neutrinos and hypothetical dark-matter particles (see also Special Feature: Sensing a Nuclear Kick on a Speck of Dust).
The basic idea is simple: if the radioactive atom is embedded in a larger object, then an outgoing decay product will exert a backreaction on that object, causing it to recoil in the opposite direction. But is it really possible to detect the recoil kick from a particle as small as a helium nucleus? The answer lies in how precisely we can measure the larger object’s momentum. One of the main limitations is friction: if the larger object is slowed down by frictional forces, then its motion won’t reflect the impulse from the decaying particle.
In this work, we show that the flexible programming of the exchange-biased magnetic heterostructure enables the direct generation of various structured terahertz beams with complex polarization distributions. In the above demonstrations, we did not perform amplitude design on ENF(r), as lasers with Gaussian profiles were utilized to excite various programmed emitters. To exert control over local NF amplitudes, spatial light modulators can be further employed to manipulate the amplitude profiles of excitation lasers.
It is important to acknowledge that, owing to the inherent capability of generating only linearly polarized ENF locally, a crucial constraint arises: the NF terahertz amplitudes for the LCP and RCP components must be equal at all locations, leading to \({A}_{NF}^{L}(\mathbf{r})={A}_{NF}^{R}(\mathbf{r})\) at the emitter’s surface. As a consequence, both LCP and RCP terahertz fields are simultaneously generated in the far field. In situations where terahertz beams with a pure polarization state are of interest, one can strategically design the magnetization pattern so that desired polarization state is focused at the center, while surrounding it with other polarizations. By employing simple spatial filtering, this pure polarization state can be isolated and utilized. This concept was demonstrated by the LCP Gaussian beam in the last demonstration, where different spatial phase gradients were applied on the LCP and RCP light beams, allowing for their spatial separation in the far field.
Furthermore, by fabricating the heterostructures into appropriately oriented micro-structures, one can induce confinements onto the local charge currents [38,39,40]. This enables independent control over the x- and y-components of the local terahertz fields, potentially facilitating the realization of an arbitrary terahertz wave generator.