Across the nation, environmentally minded scientists and engineers are leading a new generation of nuclear reactor designs. They see nuclear power as a clean, carbon-free energy source along with hydropower, wind, and solar.
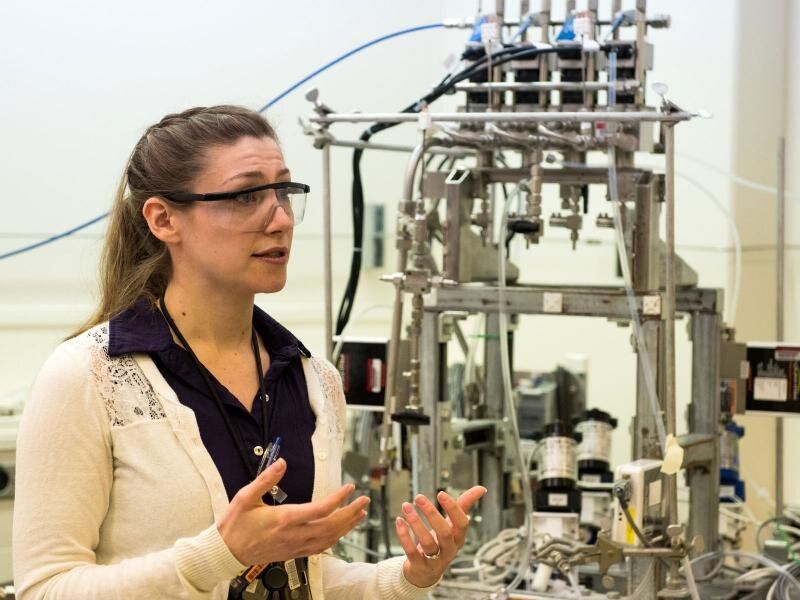
After his PhD thesis invalidates an old assumption, Norman Cao wonders what’s next.
“What are some challenges in controlling plasma and what are your solutions? What is the most effective type of fusion device? What are some difficulties in sustaining fusion conditions? What are some obstacles to receiving fusion funding?”
For the past four years, graduate student Norman Cao ’15 PhD ’20 has been the Plasma Science and Fusion Center’s (PSFC’s) go-to “answer man,” replying to questions like these emailed by students and members of the general public interested in getting a deeper understanding of fusion and its potential as a future energy source.
Energy researchers have been reaching for the stars for decades in their attempt to artificially recreate a stable fusion energy reactor. If successful, such a reactor would revolutionize the world’s energy supply overnight, providing low-radioactivity, zero-carbon, high-yield power – but to date, it has proved extraordinarily challenging to stabilize. Now, scientists are leveraging supercomputing power from two national labs to help fine-tune elements of fusion reactor designs for test runs.
In experimental fusion reactors, magnetic, donut-shaped devices called “tokamaks” are used to keep the plasma contained: in a sort of high-stakes game of Operation, if the plasma touches the sides of the reactor, the reaction falters and the reactor itself could be severely damaged. Meanwhile, a divertor funnels excess heat from the vacuum.
In France, scientists are building the world’s largest fusion reactor: a 500-megawatt experiment called ITER that is scheduled to begin trial operation in 2025. The researchers here were interested in estimating ITER’s heat-load width: that is, the area along the divertor that can withstand extraordinarily hot particles repeatedly bombarding it.
Whoever manages it first, we are on the cusp of a new age sparked by fusion giving more than it gets (producing more energy than it uses), then miniaturization for practical use and mass manufacture. That would essentially mean that we have access to an infinite, cheap, safe, and clean energy source. No more coal. No more nuclear waste. Massively less global warming. Even better, given the fact that the world runs on an energy economy built around energy scarcity, we will essentially become a post-scarcity civilization. And THAT my friends is a permanent, impossible to overstate game changer. For EVERYTHING and EVERYONE — FOREVER.
But first, scientists need to see if it’s ready.
Researchers unlocked the electronic properties of graphene by folding the material like origami paper.
Researchers at the US Department of Energy’s Princeton Plasma Physics Laboratory have created a plan using liquid lithium to control the extreme heat that could strike the exhaust system inside tokamak fusion reactors.
A tokamak is a confinement device that uses a powerful magnetic field to confine plasma in the shape of a torus and whose work is to produce controlled thermonuclear fusion power.
Fusion, on the other hand, is the nuclear reaction that occurs when atoms collide and fuse together, releasing huge amounts of energy. This process is what powers the Sun.
How much does a power system’s reliability depend on the temperature? Electric power system generator resource adequacy modeling is designed to help determine capacity requirements for electric power system operators across the United States. While calculating resource adequacy requirements has been done for a century, it requires ongoing attention as the generation mix is constantly expanding and changing. A new paper contributes to these ongoing reliability considerations by using a unique data set to determine how both low and high temperatures reduce the reliability of coal, gas, diesel, hydroelectric, and nuclear power generators and thus affect the amount of generation markets should contract for.