A groundbreaking study reveals that Alpha Centauri’s particles are already making their way into our solar system, traveling across the cosmic highway that connects star systems. These particles, ejected from the nearest stellar neighbor to Earth, could be carrying valuable insights about distant worlds and the forces that shape our galaxy.
Category: particle physics – Page 69
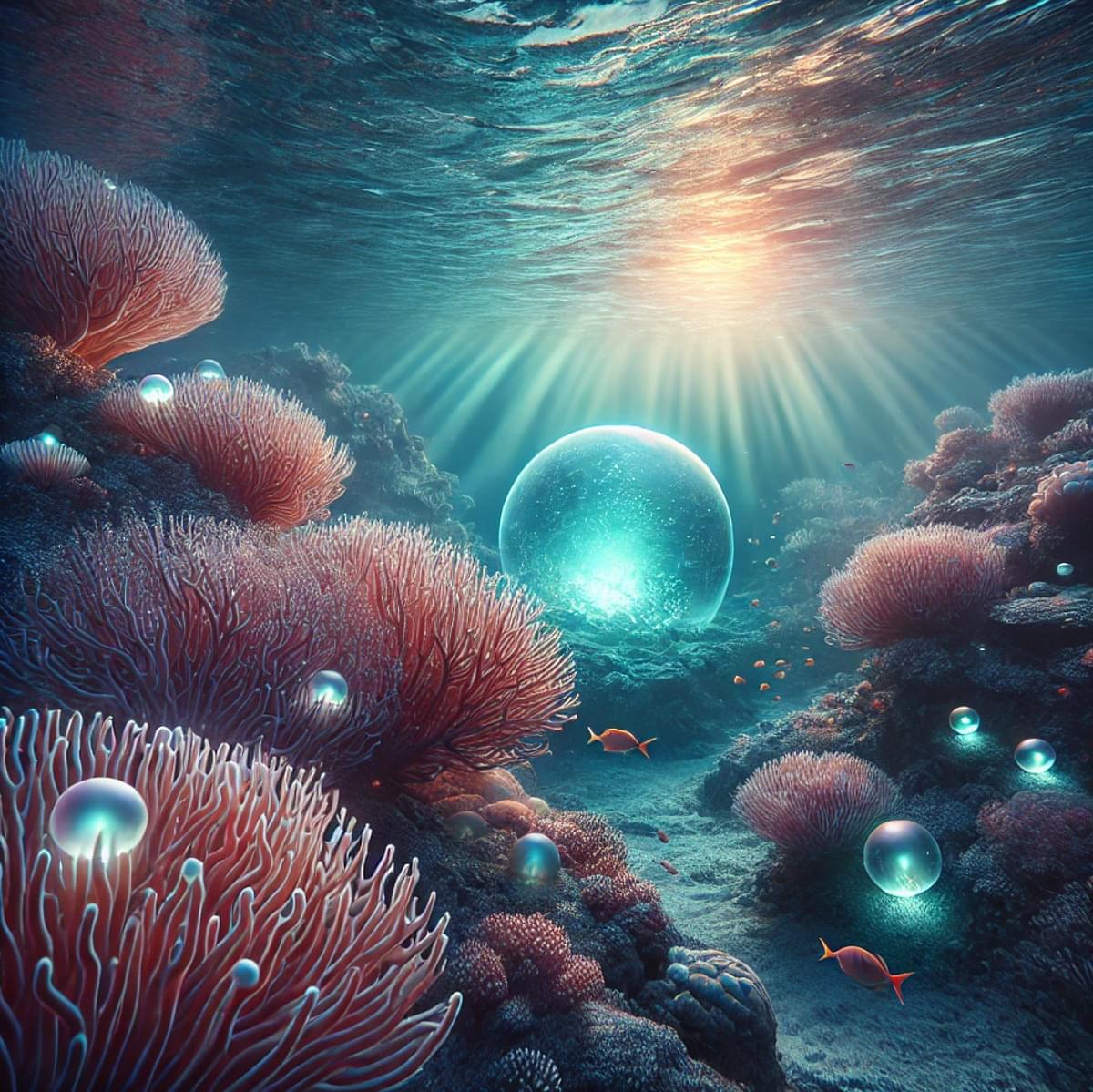
The Entangled Mind: Could Quantum Phenomena Explain Consciousness?
Read “” by Sebastian Schepis on Medium.
Imagine a world where thoughts aren’t confined to the brain, but instantly shared across a vast network of neurons, transcending the limits of space and time. This isn’t science fiction, but a possibility hinted at by one of the most puzzling aspects of quantum physics: entanglement.
Quantum entanglement, famously dubbed spooky action at a distance by Einstein, describes a phenomenon where two or more particles become intrinsically linked. They share a quantum state, no matter how far apart they are. Change one entangled particle, and its partner instantly reacts, even across vast distances.
This property, which troubled Einstein, has been repeatedly confirmed through experiments, notably by physicist John Clauser and his colleagues, who received the 2022 Nobel Prize in Physics for their groundbreaking work on quantum entanglement.
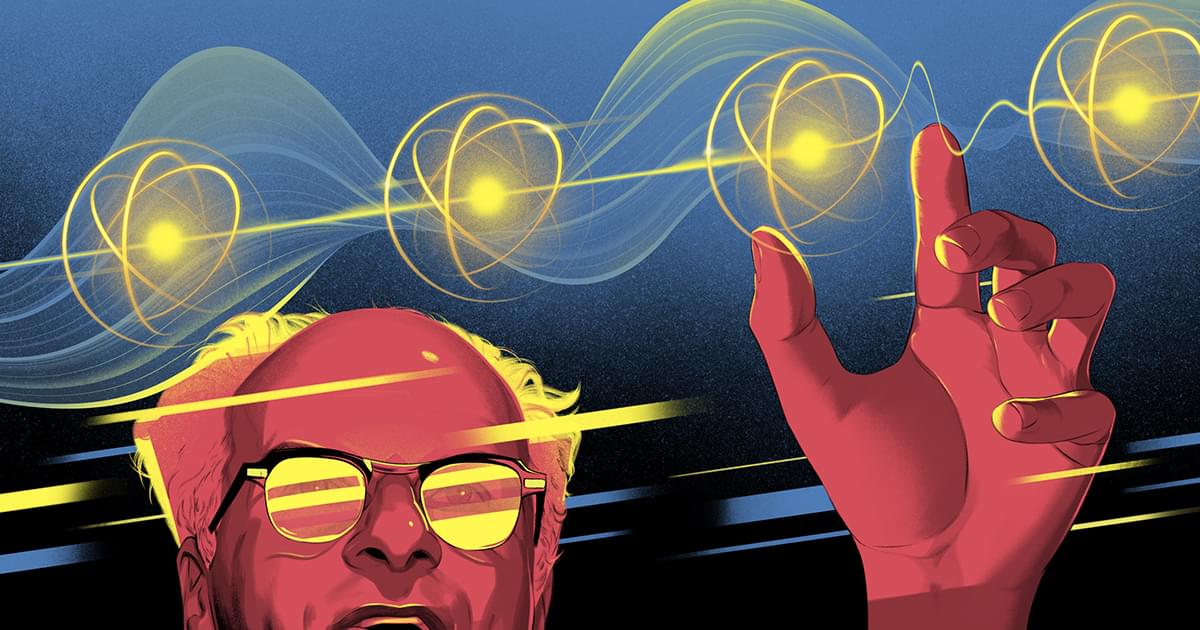
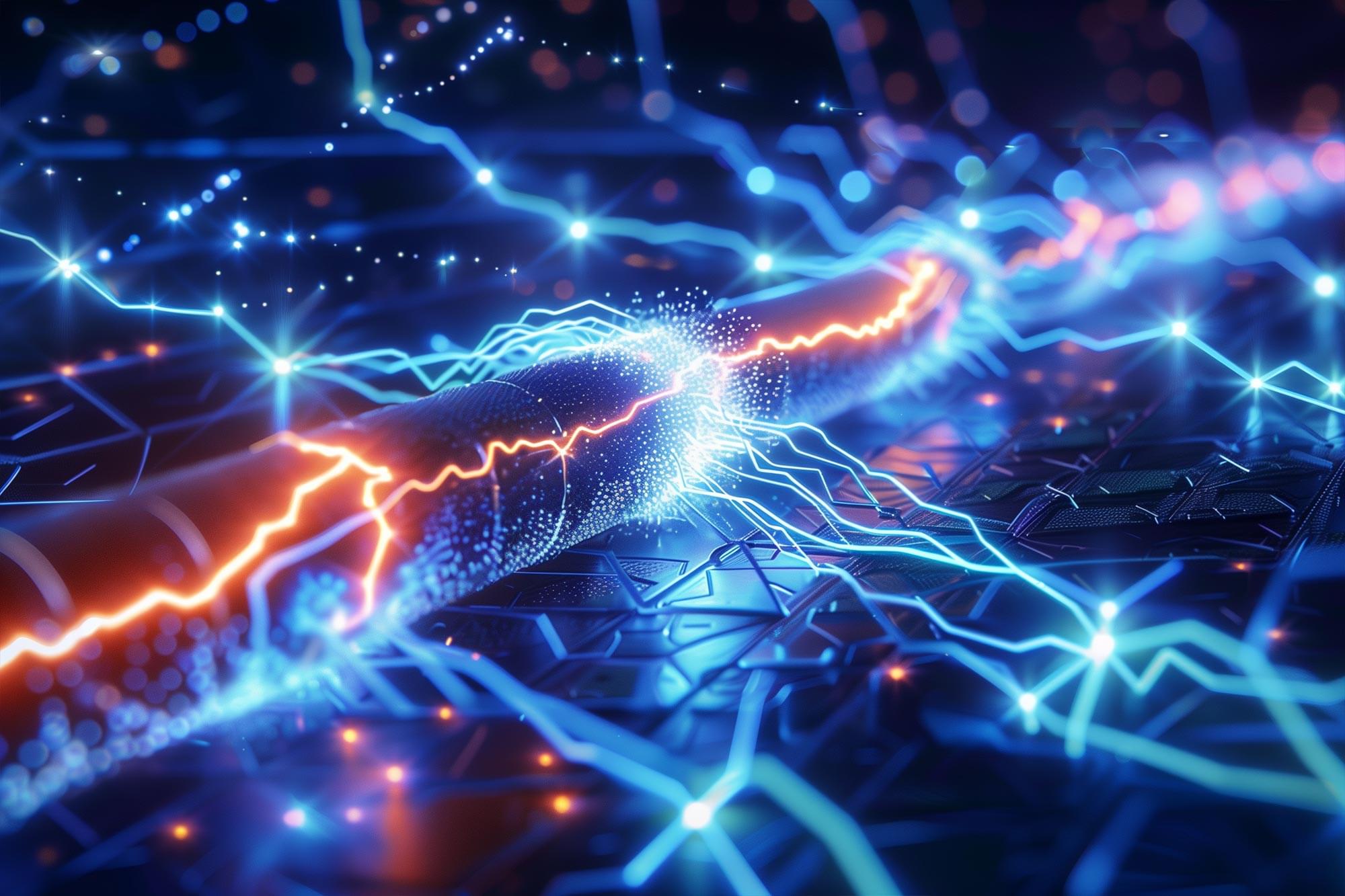
Accidental Breakthrough As Supercooled Wires Detect Near-Light-Speed Protons
Researchers have discovered that superconducting nanowire photon.
A photon is a particle of light. It is the basic unit of light and other electromagnetic radiation, and is responsible for the electromagnetic force, one of the four fundamental forces of nature. Photons have no mass, but they do have energy and momentum. They travel at the speed of light in a vacuum, and can have different wavelengths, which correspond to different colors of light. Photons can also have different energies, which correspond to different frequencies of light.

Simulations reveal Anderson transition for light in 3D disordered systems
The Anderson transition is a phase transition that occurs in disordered systems, which entails a shift from a diffusive state (i.e., in which waves or particles are spread out) to a localized state, in which they are trapped in specific regions. This state was first studied by physicist Philip W. Anderson, who examined the arrangement of electrons in disordered solids, yet it was later found to also apply to the propagation of light and other waves.
Researchers at Missouri University of Science & Technology, Yale University, and Grenoble Alpes University in France recently set out to further explore the Anderson transition for light (i.e., electromagnetic waves) in 3D disordered systems.
Their paper, published in Physical Review Letters, outlines the simulation of light wave transport in an arrangement of perfect-electric-conducting (PEC) spheres, materials that reflect electromagnetic waves.
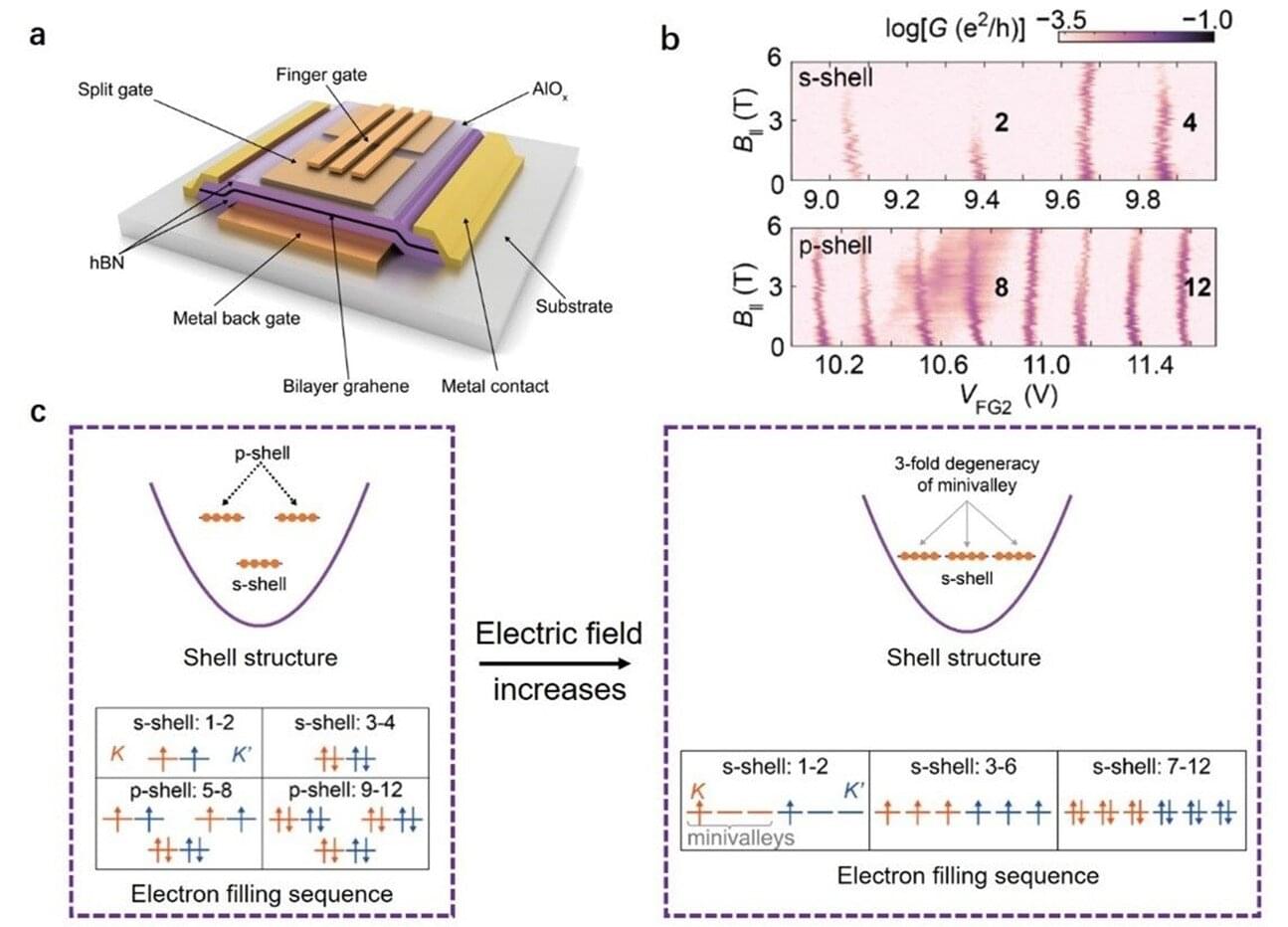
Scientists achieve electrical manipulation of spin filling sequence in bilayer graphene quantum dots
A research team from the University of Science and Technology of China has demonstrated the ability to electrically manipulate the spin filling sequence in a bilayer graphene (BLG) quantum dot (QD). This achievement, published in Physical Review Letters, showcases the potential to control the spin degree of freedom in BLG, a material with promising applications in quantum computing and advanced electronics.
BLG has drawn extensive attention in recent years due to its unique properties. When an out-of-plane electric field is applied, it can generate a tunable band gap. Moreover, the trigonal warping effect, caused by the skew interlayer coupling, gives rise to additional minivalley degeneracy, greatly influencing the behavior of charge carriers. Quantum dot devices, which can precisely control the number of charge carriers, have become a crucial tool for studying these phenomena at the single-particle level.
The research team delved into the intricate dynamics of electron shell structures within bilayer graphene quantum dot, focusing on how these structures can be manipulated through the trigonal warping effect, a unique feature of bilayer graphene. They employed a highly tunable quantum dot device, which provided the means to control the electron filling sequence. They began by applying a small perpendicular electric field, observing that the s-shell filled with four electrons, two with spin-up and two with spin-down, each from opposite valleys.
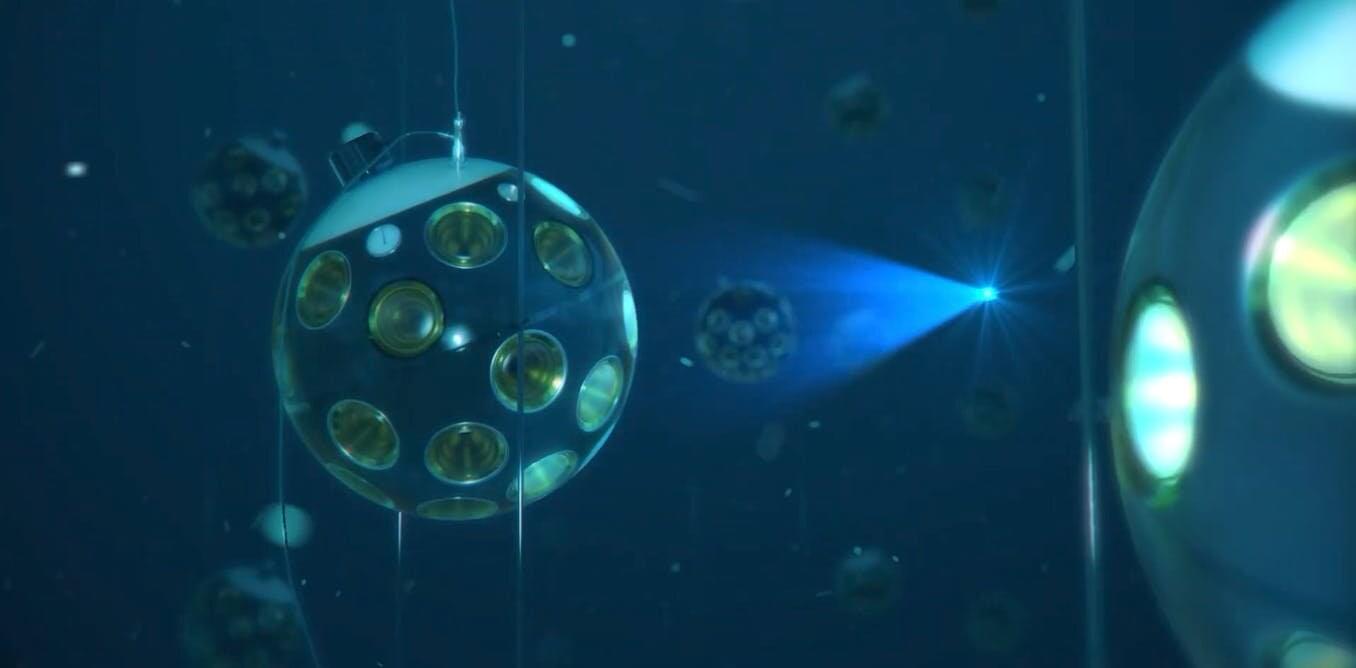
3.5 Kilometers Underwater, Scientists Found a Staggeringly Energetic Particle From Outer Space
Three and a half kilometers beneath the Mediterranean Sea, around 80km off the coast of Sicily, lies half of a very unusual telescope called KM3NeT.
The enormous device is still under construction, but today the telescope’s scientific team announced they have already detected a particle from outer space with a staggering amount of energy.
In fact, as the team report in Nature, they found the most energetic neutrino anyone has ever seen—and it represents a tremendous leap forward in exploring the uncharted waters of the extreme universe.
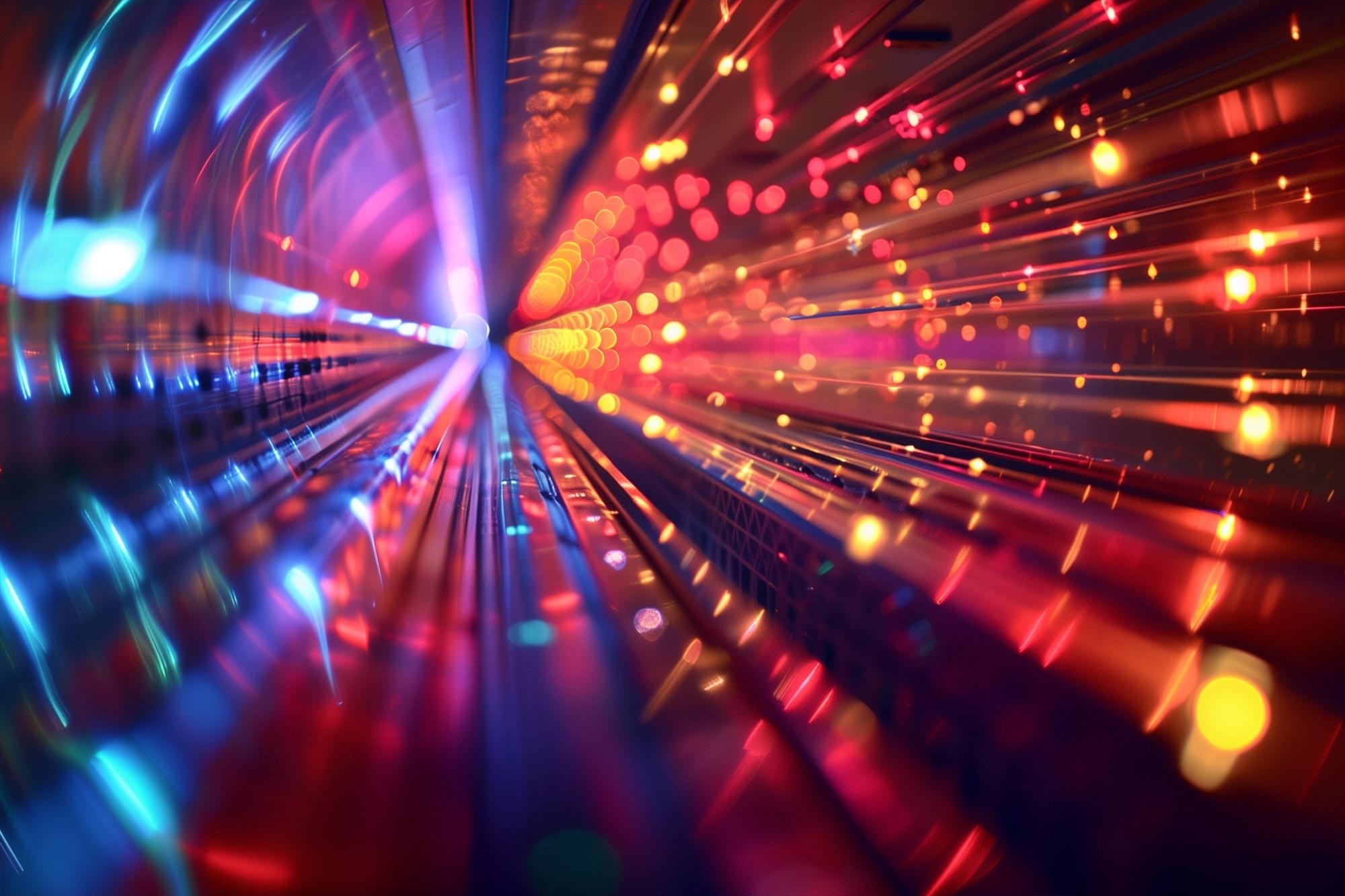
Scientists Just Unlocked a New Way to Supercharge Wireless Speeds With Graphene
Researchers have made a breakthrough in THz frequency conversion using graphene.
Graphene is an allotrope of carbon in the form of a single layer of atoms in a two-dimensional hexagonal lattice in which one atom forms each vertex. It is the basic structural element of other allotropes of carbon, including graphite, charcoal, carbon nanotubes, and fullerenes. In proportion to its thickness, it is about 100 times stronger than the strongest steel.
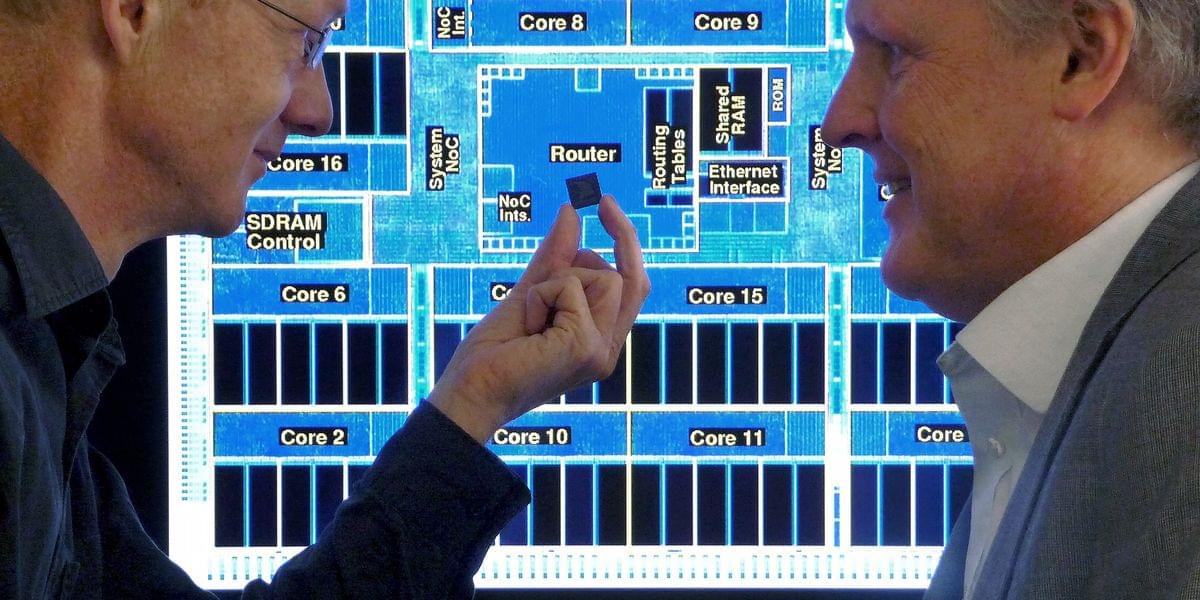
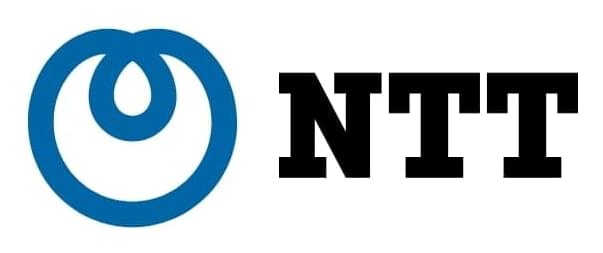