Offset your carbon footprint on Wren: https://www.wren.co/start/historyoftheuniverse The first 100 people who sign up will have 10 extra trees planted in the…
Category: particle physics – Page 218
How Does The Nucleus Hold Together?
Check out http://rocketmoney.com/pbsspace or scan the QR code on the screen to start managing your personal finances today. Thank you to Rocket Money for sponsoring today’s video! #rocketmoney #personalfinance.
PBS Member Stations rely on viewers like you. To support your local station, go to: http://to.pbs.org/DonateSPACE
Sign Up on Patreon to get access to the Space Time Discord!
https://www.patreon.com/pbsspacetime.
Two protons next to each other in an atomic nucleus are repelling each other electromagnetically with enough force to lift a medium-sized labradoodle off the ground. Release this energy and you have, well, you have a nuclear explosion. Just as well there’s an even stronger force than the electromagnetism holding our nuclei together. But it’s not the strong force, as you might have imagined. At least not directly. Nuclei are held together by a quirk of nature, without which we would have no complex atoms, no chemistry, and certainly no labradoodles.
Episode Companion Playlist.
Check out the Space Time Merch Store.
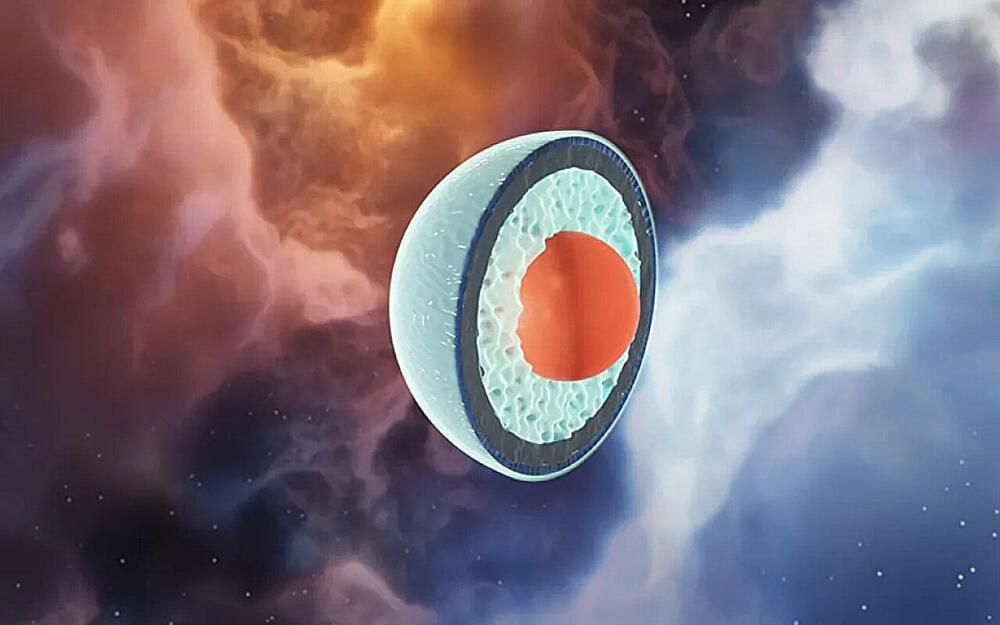
Further evidence for quark-matter cores in massive neutron stars
Neutron-star cores contain matter at the highest densities reached in our present-day universe, with as much as two solar masses of matter compressed inside a sphere of 25 km in diameter. These astrophysical objects can indeed be thought of as giant atomic nuclei, with gravity compressing their cores to densities exceeding those of individual protons and neutrons many-fold.
These densities make neutron stars interesting astrophysical objects from the point of view of particle and nuclear physics. A longstanding open problem is whether the immense central pressure of neutron stars can compress protons and neutrons into a new phase of matter, known as cold quark matter. In this exotic state of matter, individual protons and neutrons no longer exist.
“Their constituent quarks and gluons are instead liberated from their typical color confinement and are allowed to move almost freely,” explains Aleksi Vuorinen, professor of theoretical particle physics at the University of Helsinki.
Quantum Entanglement Explained — How does it really work?
To learn QM or quantum computing in depth, check out: https://brilliant.org/arvinash — Their course called “Quantum computing” is one of the best. You can sign up for free! And the first 200 people will get 20% off their annual membership. Enjoy!
Chapters:
0:00 — Weirdness of quantum mechanics.
1:51 — Intuitive understanding of entanglement.
4:46 — How do we know that superposition is real?
5:40 — The EPR Paradox.
6:50 — Spooky action and hidden variables.
7:51 — Bell’s Inequality.
9:07 — How are objects entangled?
10:03 — Is spooky action at a distance true?
10:40 — What is quantum entanglement really?
11:31 — How do two particles become one?
13:03 — What is non locality?
14:05 — Can we use entanglement for communication?
15:08 — Advantages of quantum entanglement.
15:49 — How to learn quantum computing.
Summary:
Albert Einstein described Entanglement as “spooky action at a distance,” where doing something to one of a But it’s not spooky action at a distance, at all. So what is entanglement?
Electrons have a quantum property called spin that makes them act like little magnets. We’ll always measure it pointing in one direction or the opposite: up or down, say. If we entangle two electrons so that their spins are always pointing in opposite directions, the two spins are said to be correlated. If we entangle the two electrons in this way – and fire them in opposite directions, we don’t know which one of the pair is up and which one is down until we make a measurement. If we find that electron 1 is spin up. We know the spin of electron 2 must be down.
Why isn’t this like a pair of gloves? The handedness of the gloves is there from the start. It never changes. With entangled particles that’s not the case. They are in a superposition. Prior to measurement, there is no definite answer.
How do we know superposition is real? The double slit experiment is good evidence. Entangled particles are stranger, because a measurement on one particle determines the outcome for both of them.
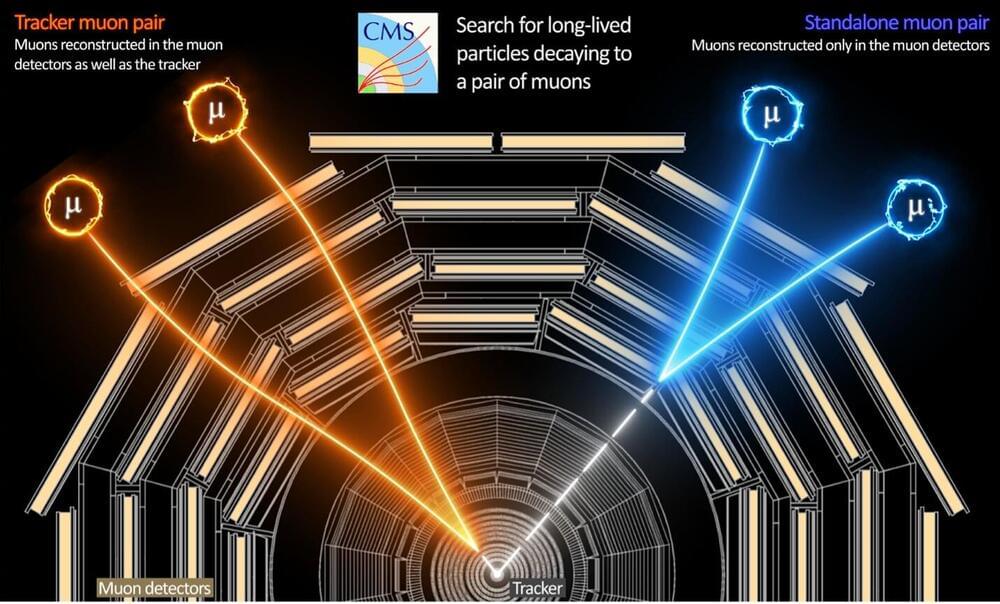
Latest search for new exotic particles at CERN
The CMS experiment has presented its first search for new physics using data from Run 3 of the Large Hadron Collider. The new study looks at the possibility of “dark photon” production in the decay of Higgs bosons in the detector.
Dark photons are exotic long-lived particles: “Long-lived” because they have an average lifetime of more than a tenth of a billionth of a second—a very long lifetime in terms of particles produced in the LHC—and “exotic” because they are not part of the standard model of particle physics.
The standard model is the leading theory of the fundamental building blocks of the universe, but many physics questions remain unanswered, and so searches for phenomena beyond the standard model continue. CMS’s new result defines more constrained limits on the parameters of the decay of Higgs bosons to dark photons, further narrowing down the area in which physicists can search for them.
Finding meaning at the quantum level
Kmele steps inside Fermilab, America’s premiere particle accelerator facility, to find out how the smallest particles in the universe can teach us about its biggest mysteries.\
\
This video is an episode from @The-Well, our publication about ideas that inspire a life well-lived, created with the @JohnTempletonFoundation.\
\
Watch the full podcast now ► • Dispatches from The Well \
\
According to Fermilab’s Bonnie Flemming, the pursuit of scientific understanding is “daunting in an inspiring way.” What makes it daunting? The seemingly infinite number of questions, with their potentially inaccessible answers.\
\
In this episode of Dispatches from The Well, host Kmele Foster tours the grounds of America’s legendary particle accelerator to discover how exploring the mysteries at the heart of particle physics help us better understand some of the most profound mysteries of our universe.\
\
Read the video transcript ► https://bigthink.com/the-well/dispatc…\
\
00:00:00 — The Miracle of Birth\
00:04:48 — Exploring the Universe’s Mysteries\
00:09:20 — Building Blocks of Matter and the Standard Model\
00:13:35 — The Evolving Body of Knowledge\
00:17:39 — Understanding the Early Universe\
00:22:05 — Reflections on Particle Physics\
00:25:34 — The Extraordinary Effort to Understand the Small\
00:29:59 — From Paleontology to Astrophysics\
00:33:40 — The Importance of the Scientific Method and Being Critical\
\
\
About Kmele Foster:\
\
Kmele Foster is a media entrepreneur, commentator, and regular contributor to various national publications. He is the co-founder and co-host of The Fifth Column, a popular media criticism podcast.\
\
He is the head of content at Founders Fund, a San Francisco based venture capital firm investing in companies building revolutionary technologies, and a partner at Freethink, a digital media company focused on the people and ideas changing our world.\
\
Kmele also serves on the Board of Directors of the Foundation for Individual Rights and Expression (FIRE).\
\
\
Read more from The Well: \
Actually, neuroscience suggests “the self” is real\
► https://bigthink.com/the-well/actuall…\
Mary Shelley’s Frankenstein can illuminate the debate over generative AI\
► https://bigthink.com/the-well/mary-sh…\
Few of us desire true equality. It’s time to own up to it\
► https://bigthink.com/the-well/few-des…\
\
\
About The Well\
Do we inhabit a multiverse? Do we have free will? What is love? Is evolution directional? There are no simple answers to life’s biggest questions, and that’s why they’re the questions occupying the world’s brightest minds.\
\
Together, let’s learn from them.\
\
\
\
Join The Well on your favorite platforms:\
► Facebook: https://bit.ly/thewellFB \
► Instagram: https://bit.ly/thewellIG
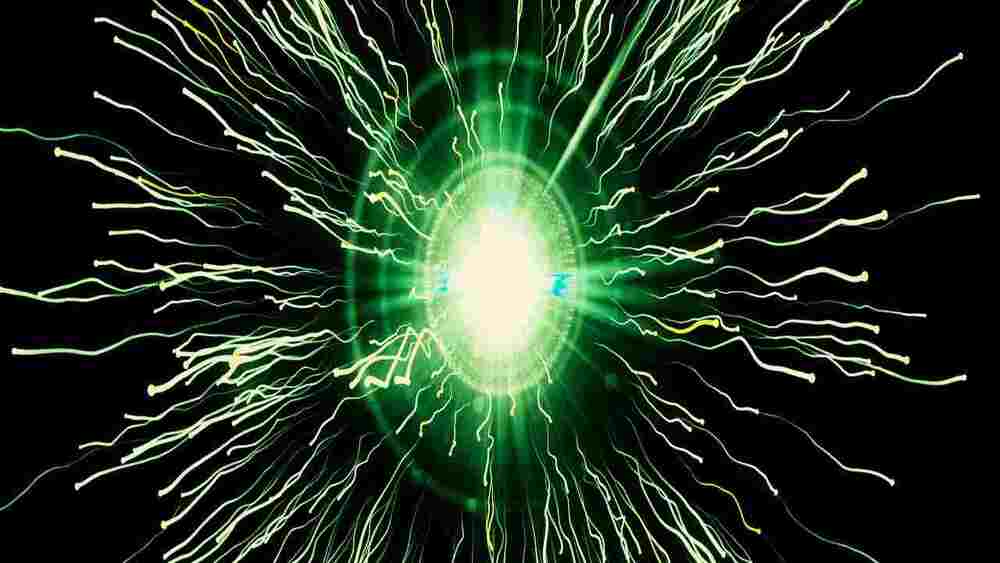
How A Laser-Plasma Collider Can Turn Light Into Matter In A Matter Of Seconds
A team led by researchers at Osaka University and University of California, San Diego has conducted simulations of creating matter solely from collisions of light particles. Their method circumvents what would otherwise be the intensity limitations of modern lasers and can be readily implemented by using presently available technology. This work might help experimentally test long-standing theories such as the Standard Model of particle physics, and possibly the need to revise them.
One of the most striking predictions of quantum physics is that matter can be generated solely from light (i.e., photons), and in fact, the astronomical bodies known as pulsars achieve this feat. Directly generating matter in this manner has not been achieved in a laboratory, but it would enable further testing of the theories of basic quantum physics and the fundamental composition of the universe.
In a study published in Physical Review Letters, a team led by researchers at Osaka University has simulated conditions that enable photon–photon collisions, solely by using lasers. The simplicity of the setup and ease of implementation at presently available laser intensities make it a promising candidate for near-future experimental implementation.
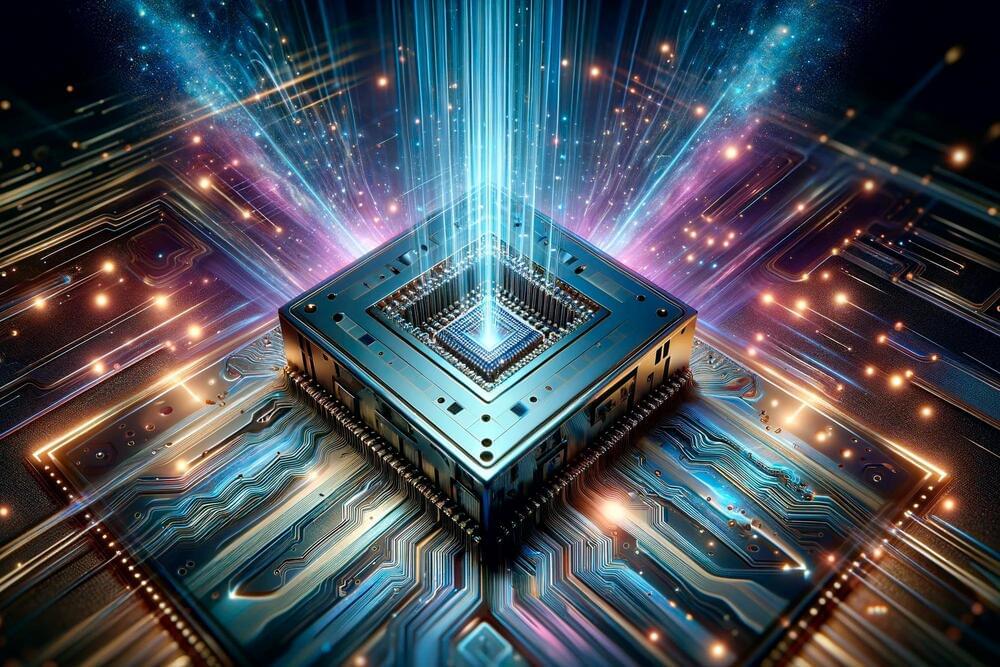
Harvard Unveils World’s First Logical Quantum Processor
Harvard’s breakthrough in quantum computing features a new logical quantum processor with 48 logical qubits, enabling large-scale algorithm execution on an error-corrected system. This development, led by Mikhail Lukin, represents a major advance towards practical, fault-tolerant quantum computers.
In quantum computing, a quantum bit or “qubit” is one unit of information, just like a binary bit in classical computing. For more than two decades, physicists and engineers have shown the world that quantum computing is, in principle, possible by manipulating quantum particles – be they atoms, ions or photons – to create physical qubits.
But successfully exploiting the weirdness of quantum mechanics for computation is more complicated than simply amassing a large-enough number of physical qubits, which are inherently unstable and prone to collapse out of their quantum states.
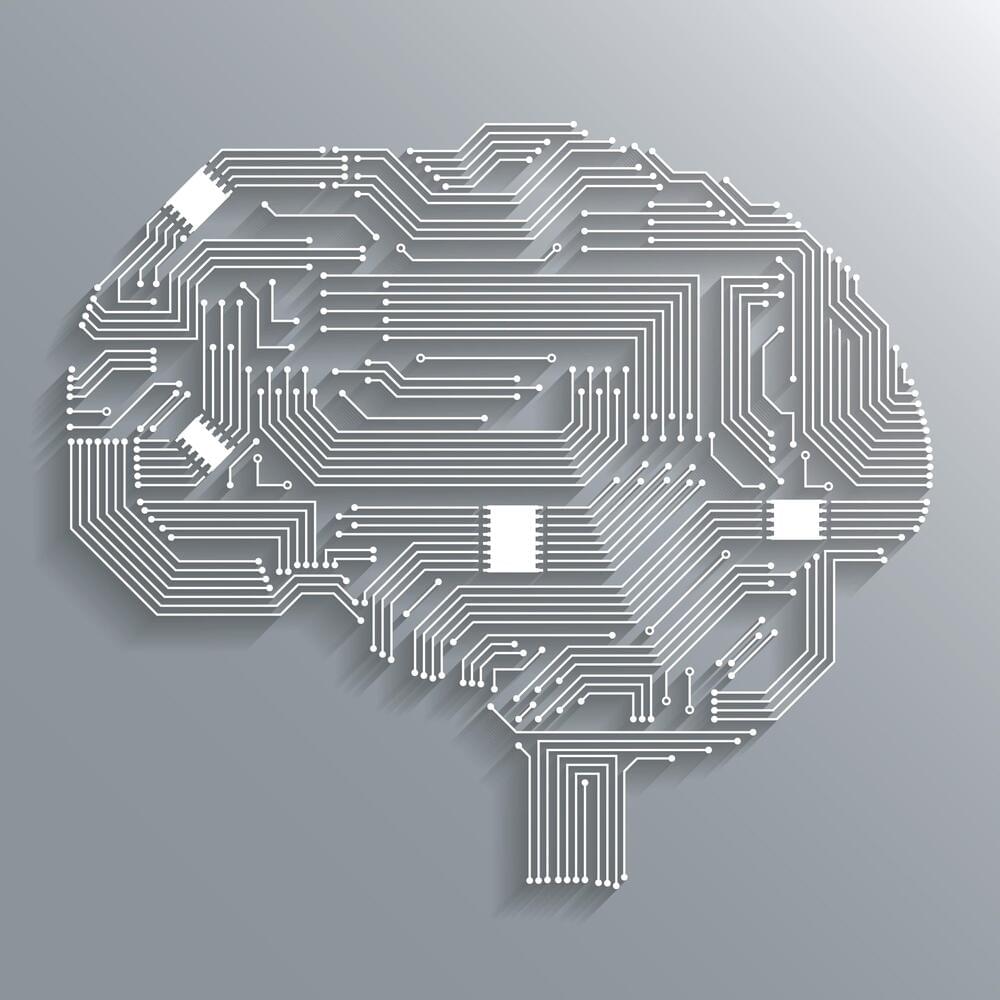
This Machine Learning Research Opens up a Mathematical Perspective on the Transformers
The release of Transformers has marked a significant advancement in the field of Artificial Intelligence (AI) and neural network topologies. Understanding the workings of these complex neural network architectures requires an understanding of transformers. What distinguishes transformers from conventional architectures is the concept of self-attention, which describes a transformer model’s capacity to focus on distinct segments of the input sequence during prediction. Self-attention greatly enhances the performance of transformers in real-world applications, including computer vision and Natural Language Processing (NLP).
In a recent study, researchers have provided a mathematical model that can be used to perceive Transformers as particle systems in interaction. The mathematical framework offers a methodical way to analyze Transformers’ internal operations. In an interacting particle system, the behavior of the individual particles influences that of the other parts, resulting in a complex network of interconnected systems.
The study explores the finding that Transformers can be thought of as flow maps on the space of probability measures. In this sense, transformers generate a mean-field interacting particle system in which every particle, called a token, follows the vector field flow defined by the empirical measure of all particles. The continuity equation governs the evolution of the empirical measure, and the long-term behavior of this system, which is typified by particle clustering, becomes an object of study.
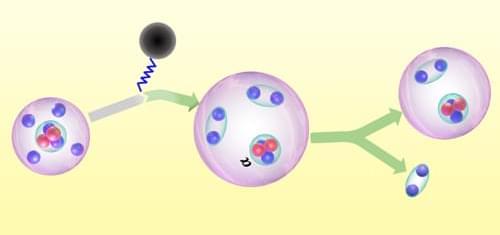
Neutron Pairs Condense in Excited Helium-8
In its ground state, the helium-8 (8He) nucleus consists of an alpha particle (4He nucleus) and four neutrons. If, before its few-hundred-milliseconds life ends, an 8 He nucleus is nudged into its first 0+ excited state, the four neutrons form two pairs known as dineutron clusters. According to theory, the alpha particle and the two neutron clusters settle into a three-member nuclear analog of a Bose-Einstein condensate. That outcome has now been observed for the first time by Zaihong Yang of Peking University and his colleagues at the RIKEN Nishina Center in Japan [1].
The experiment entailed firing a high-intensity beam of 8 He nuclei at polyethylene and carbon targets. Some collisions excited the nuclei into the sought-after condensate state, which promptly broke up into a helium-6 (6He) nucleus and a single neutron pair. The 6 He nuclei made their way through dipole magnets to drift detectors and plastic scintillators for characterization. The neutrons struck a plastic scintillator whose layered construction made it possible to identify which neutrons were correlated—that is, members of a dineutron cluster—and which were not. The correlated neutron pairs and the scattering count rate’s dependence on energy, angle, and type of target were all consistent with theoretical predictions of the nature of the correlated 8 He excited state.
The 8 He condensate resembles the so-called Hoyle state of carbon-12, which consists of three alpha particles in the condensed state. Astronomer Fred Hoyle predicted the state in 1954 to account for the synthesis of carbon in helium-burning stars. Yang points out that nuclear condensates could also have implications for understanding the structures of exotic nuclei and neutron stars.